Weighty thoughts on antimatter
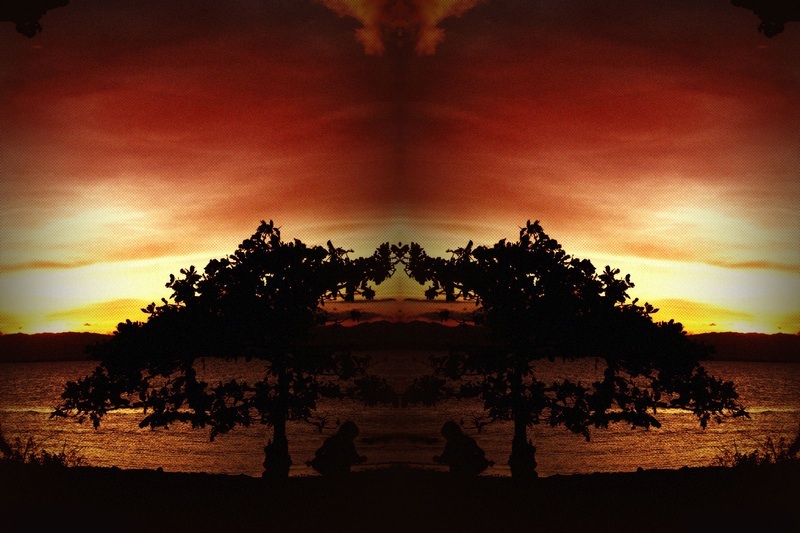
A pulse of particles speeds into the vacuum chamber. Positrons, 20,000,000 antimatter particles, clumped in a pulse one nanosecond deep. Like a silent, angry swarm they are targeted into a porous silica target. The positrons are confined by a magnetic field, increasing their interaction with the silica. Some attract electrons and synthesize into positronium, a hybrid unstable ‘molecule’. Before it can decay the positronium is excited first by a burst of ultra-violet laser light. Then a second burst, this time in the infrared. With each excitation the positronium puffs-up. The still bound, positron and electron orbit further and further away from each other. Decreasing their opportunity to meet. Increasing the lifetime of the positronium. Eventually they meet. They annihilate. Mutually destructing in a soundless flash of energy. A pair of gamma rays to remind us of a nanosecond long courtship.
Producing positronium, binding the negatively charged electron with its antimatter equivalent, the positively charged positron, is not a normal occurrence in our world. If this experiment sounds a little like science fiction, the reason for doing it is part of an even more exotic tale. This laboratory experiment is to produce ‘long-lived’ positronium is so that the researchers can measure the effect of gravity on it. Published in January 2012, this success represents 10 years of hard work for Allen Mills and his team from the University of California, Riverside.
The positron is the antimatter version of the electron. It has the identical mass to the electron, but a positive charge. The researchers are trying to find whether matter and antimatter behave the same way under gravity. That is, do they weigh differently? If they find such behaviour it would truly rock the physics world.
Antimatter. The idea of it is weird. The idea of weighing it is stranger still.
The weirdness of antimatter
Antimatter has been one of the most fascinating fields of research ever since the prediction of its existence by Paul Dirac in 1929. Paul Dirac solved the basic equations of quantum mechanics. In doing so he found their solution implied the existence of antimatter particles. At the time most physicists, Dirac included, thought the idea was preposterous.
Within a short time, experimenters looking at cosmic rays bombarding the Earth found particles that behaved like electrons, but with a positive charge. In 1932 Carl Anderson carried out the definitive experiments demonstrating the existence of the anti-electron, naming it the positron, and being awarded the Nobel Prize in 1936 for his discovery.
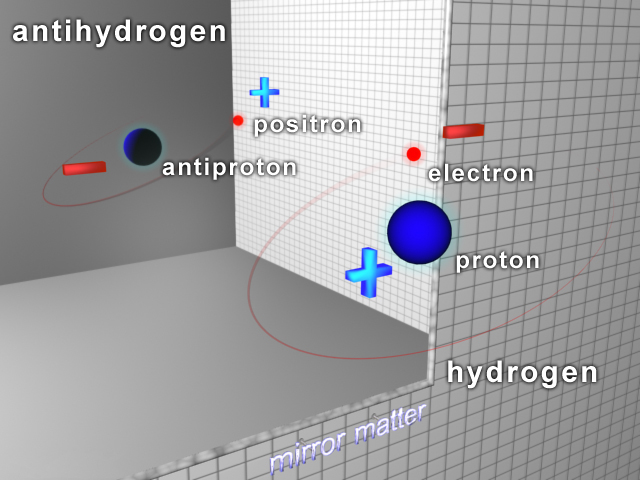
Dirac had postulated in 1931 that each of the fundamental particles has an equivalent antimatter partner. Furthermore, when matter and its antimatter opposite come into contact they annihilate each other, releasing energy into two equal energy photons. Their energy is given by Einstein’s famous equation E=mc². This latter relationship has been much exploited by science fiction writers. Matter – antimatter reactions powered the star-ship USS Enterprise in the 60s hit TV show Star Trek.
Work with high-energy antiparticles is now commonplace in physics and materials science. Anti-electrons are used regularly in the medical technique of positron emission scanning tomography. Perhaps more importantly, the equivalence model of matter and antimatter has been fully incorporated into the Standard Model of Particle Physics. Matter particles and their anti-matter pairs have the same mass and equal, but opposite charge.
The most important point in all of this focusses on when our universe came into being, the Big Bang. Models of the Big Bang predict that equal amounts of matter and antimatter were formed initially. Ordinary matter is clearly what our observable Universe is made of today. Where is the antimatter? A glaring, niggling, imbalance that begs for an explanation.
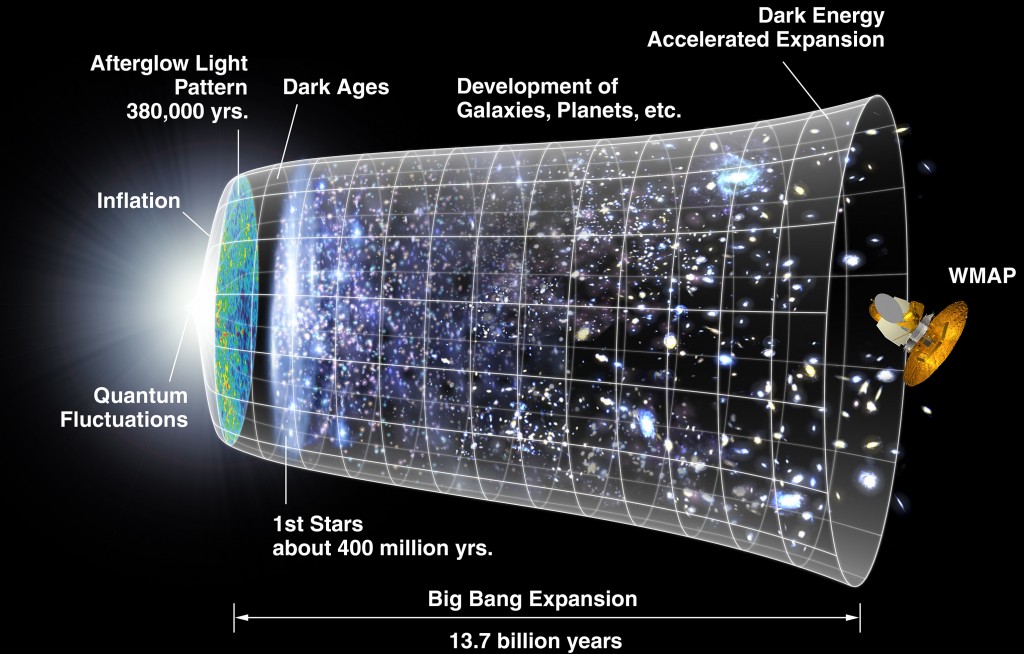
This imbalance could be explained by a slight difference in one of the fundamental properties of particle-antiparticle pairs (such as charge or mass). There isn’t yet any experimental evidence for such a difference. Another alternative might be a difference in their gravitational attractiveness. It is widely expected that the gravitational interaction of matter and antimatter should be identical. If they were not, then this could explain the preponderance of matter in our universe.
Elementary antimatter particles naturally occur in radioactive decays and in cosmic radiation. Some of them, such as the positron and the antiproton, have been studied extensively and even compared to their matter equivalents. Measurements with charged antiparticles are difficult because gravity is a far weaker force than the electromagnetic force. The first experiments to measure the gravitational attraction of antimatter were at the University of Stanford and CERN in 1974 and 1993 respectively. Both used charged antimatter particles. The experiments were marred by stray electric fields and did not produce satisfactory results.
Trying to do experiments like this requires precision and reproducible backgrounds. The world is a rather messy place to measure these relationships. Neutral positronium, such as in Mill’s experiments, or an anti-atom could be used to test the effect of gravity on antimatter for the first time, because it is immune to stray electromagnetic fields that have hampered the previous studies with charged antimatter particles.
Does antimatter fall down?
Understanding gravity has proven to be a little more complicated than falling apples. To the Greek philosopher and polymath Aristotle, the concept that heavy objects fell faster than light objects was obvious. His elegant, but fanciful, notion persisted well into the Middle Ages. The Italian physicist, mathematician, and astronomer Galileo Galilei successfully challenged Aristotle’s impractical theories of motion. Experiments using the swing of pendulums proved him correct. Observing their swing rates was more practical than the dropping of objects from the Leaning Tower of Pisa, as Galileo had originally proposed.
Galileo also put forward the basic principle of relativity, that the laws of physics are the same in any system that is moving at a constant speed in a straight line, regardless of its particular speed or direction. Hence, there is no absolute motion or absolute rest. This principle is central to Einstein’s special theory of relativity.
The key point under consideration here is the correlation between inertial mass and gravitational mass. This is the correlation between the forces measured on a mass when it is falling under gravity or being accelerated. The earliest experiments were done by English natural philosopher Isaac Newton and improved upon by the German mathematician and astronomer Friedrich Wilhelm Bessel in the 1820s.
The problem is that Newton’s theories and his mathematical formulae did not and do not explain the equivalence of the behavior of various masses under the influence of gravity, independent of the quantities of matter involved. The observation that the gravitational mass and the inertial mass is the same for all objects is unexplained within Newton’s Theories. The experiments of Galileo Galilei, decades before Newton, established that objects that have the same air or fluid resistance are accelerated by the force of the Earth’s gravity equally, regardless of their different inertial masses. Yet, the forces and energies that are required to accelerate various masses is completely dependent upon their different inertial masses, as can be seen from Newton’s Second Law of Motion, F = ma.
Precision measurements by the Hungarian physicist Loránd Eötvös originally in 1885, and then again with improved instruments and precision between 1906 and 1909, established the universality of Newton’s law of gravitation. These were followed with a series of similar but more accurate experiments, these included experiments with different types of materials, on moving ships and in different locations around the Earth. These experiments demonstrated the equivalence of gravitational and inertial mass for ordinary matter. In turn, these experiments led to the modern understanding of the equivalence principle encoded in general relativity, which states that the gravitational and inertial masses are the same.
So far, so good, for understanding the behaviour of ordinary matter. Rather than disembodied logic alone, the combination of experiment, measurement, and sound reasoning proving to be the correct way to discern the laws that represent reality.
Gravity is now best described by general relativity. General relativity is a classical theory that does not imply the existence of antimatter. In the 1980s a quantum-mechanical formulation of gravity allowed for non-Newtonian contributions to the force which might lead to a difference in the gravitational force on matter and antimatter.
A number of theories propose how differential interactions between matter and antimatter may be explained. It must also be pointed out that numerous models and experiments with matter have been used to derive upper limits on the possible differences in the nature of such gravitational attractions.
Direct investigation of antimatter, experimentally, is characterised by an almost complete lack of data.
The experimenters
Allen Mills is not alone in his quest to measure the weight of antimatter.
In 2011 the AEgIS collaboration at CERN, had funding approved to use antihydrogen to measure any difference in the gravitational force on matter and antimatter. CERN is Europe’s particle-physics research lab located near Geneva in Switzerland. Perhaps currently best known for its search for the Higgs boson, the co-called God particle. The funding scale of the AEgIS experiment (Antimatter Experiment: Gravity, Interferometry, Spectroscopy) is more modest. It’s goal, to create a horizontal beam of antihydrogen and to study its free fall in the Earth’s gravitational field with a matter wave interferometry apparatus, is scientifically equally far-reaching.
The quest to create, trap and study antihydrogen is now entering its third decade at CERN.
In 2002, the ATHENA experiment at CERN’s Antiproton Decelerator was the first to produce copious amounts of cold antihydrogen, the simplest atomic antimatter system. The ATHENA (AnTiHydrogEN Apparatus) experiment had the objective to produce, to store and to study antihydrogen at extremely low temperatures, at less than 1 Kelvin temperature. The goal was to compare the energy levels of antihydrogen and hydrogen with extreme accuracy. The ATHENA set-up was used as a proof of concept in this case for the successor experiment AEgIS.
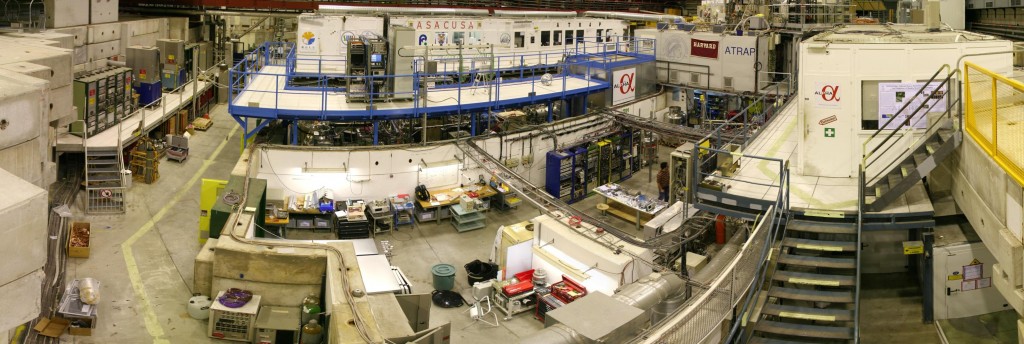
The antiprotons supplied by the Antiproton Decelerator were trapped and cooled, and brought into overlap with positrons from a radioactive sodium source in a cylindrical Penning trap. The produced anti-atoms, no longer confined in the charged-particle trap, drifted radially outward and annihilated on the electrodes. ATHENA’s sophisticated detector allowed the temporally and spatially resolved reconstruction of these annihilation events.
During the data taking periods in 2003 and 2004, the experimental parameters were optimized in order to maximize the antihydrogen production rate, and the temperature and internal quantum states of the anti-atoms were determined. ATHENA was not configured to measure the gravitational attraction of antihydrogen. Data taking with ATHENA has now ended.
It was collaborators from the ATHENA experiment, along with new groups from other institutes, that have designed the successor experiment, AEgIS, with the aim of performing gravitational studies with antimatter. The AEgIS proposal was submitted in January 2008 and approved by the CERN Research Board in December 2008. Construction began in early 2010.
Meanwhile the CERN group has been building on its expertise for the production and trapping of antihydrogen. A new experimental collaboration called ALPHA (Antihydrogen Laser PHysics Apparatus) is another successor to ATHENA. In late 2010 the ALPHA group managed, 38 times to confine single antihydrogen atoms for 172 milliseconds. At the time the spokesperson Jeffrey Hangst said, “We’re ecstatic. This is five years of hard work.”
By July 2011 they had confined seven antihydrogen anti-atoms for 1,000 seconds, extending their earlier results by nearly four orders of magnitude. To compare with these CERN successes, Mills, in his late 2011 experiment, produced 12 positronium atoms that did not annihilate until they hit the chamber wall. This journey of a few centimetres takes about a microsecond.
Based on these results Mills believes he can produce a collimated, long-lived beam for the direct measurement of the gravitational free fall of positronium atoms.
Kudos and plaudits
We have in 2012 then, two experiments, both different in their experimental make-up. Both are trying to measure the gravitational free-fall of antimatter: one using antihydrogen, one using positronium. Assuming that both will be successful, then one will be used as a confirmation of the results of the other.
This is how great science is done. Great scientists are nonetheless people. People are competitive. In years to come, the science textbooks will record, and perhaps laud, who was first to measure the weight of antimatter.
Great article, Kevin!
Entertaining and informative. Extremely well written and thoughtful. A fantastic introduction for the layperson to antimatter and it’s importance in our universe, through scientific history and today. But also great for the science buffs and professionals.
These are definitely two experiments worth monitoring! Exciting times. I hope you follow up this thread as progress is, hopefully, made … the contest is clearly on!
Thanks Viviane, for the feedback and encouragement. I will be following these experiments as they progress…and also other interesting ones on antimatter.